Extracorporeal shockwave treatment: A novel tool to improve Schwann cell isolation and culture
Background aims
As new approaches for peripheral nerve regeneration are sought, there is an increasing demand for native Schwann cells for in vitro testing and/or reimplantation. Extracorporeal shockwave treatment (ESWT) is an emergent technology in the field of regenerative medicine that has also recently been shown to improve peripheral nerve regeneration.
Methods. In this study, we elucidate the effects of ESWT on Schwann cell isolation and culture. Rat sciatic nerves were dissected and treated with ESWT, and Schwann cells were isolated and cultured for 15 passages. Results. Single treatment of the whole nerve ex vivo led to significantly increased extracellular adenosinetriphosphate as an immediate consequence, and subsequently a number of effects on the culture were observed, starting with a significantly increased Schwann cell yield after isolation. In the ESWT group, the quality of culture, reflected in consistently higher purity (S100b, morphology), proliferation rate (5-bromo-2-deoxyuridine, population doublings per passage) and expression of regenerative phenotype- associated markers (P75, glial fibrillary acidic protein, c-Jun), was significantly improved. In contrast, the control group exhibited progressively senescent behavior, reflected in a decrease of proliferation, loss of specific markers and increase in P16INK4A expression.
Background
Peripheral nerve lesions occur with an incidence of
approximately 300 000 cases annually in Europe, representing a frequent cause of hospitalization and displaying a major burden to patients and social health care [1]. Although the peripheral nerve system has a remarkable regenerative potential, regeneration over nerve gaps or over long distances (e.g., after proximal lesions) presents several difficulties. In this regard, nerve autografts are the gold standard to treat peripheral nerve injuries with tissue loss but often do not
result in a satisfactory outcome [2]. In particular, long-distance gaps or severe injuries affecting several nerves push autografting to its limits regarding the availability of donor material. Alternatives to facilitate nerve
regeneration, such as artificial nerve guidance tubes
or other types of scaffolds or application of neurotrophic substances, are sought. Some of these approaches are currently used in clinical nerve repair, although there is an ongoing debate concerning their appropriate use, effectiveness and side effects [3,4]. One of the major reasons for the unsatisfactory outcome after repair of long-distance gaps is the limited
proliferative capacity of Schwann cells [5]. Schwann
cells play a key role in peripheral nerve regeneration: they participate in the removal of myelin and axonal remnants, start proliferation and align to build the so-called bands of Büngner [6]. After the axon has elongated along the bands of Büngner, the Schwann cells start to remyelinate the newly formed axon to complete the regenerative process. A novel strategy to improve the functional outcome of peripheral nerve regeneration is the therapy of injured nerves with extracorporeal shockwave treatment (ESWT). ESWT has its origin in the field of urology, in which it is used to destroy kidney stones [7], but it has also been proven to be an effective therapeutic tool in the field of regenerative medicine. In
preclinical and clinical trials, beneficial effects have been
reported in treatment of various medical indications such as non-union fractures [8–10], ischemia-induced tissue necrosis [11], or chronic wounds [12,13]. The shockwave generated is a sonic pulse and is characterized by an initial rise, reaching a positive peak of up to 100 MPa within 10 ns, followed by a negative amplitude of up to −10 MPa and a total life cycle of
less than 10 μs. Biological responses are thought to be triggered by the high initial pressure, followed by a tensile force and the resulting mechanical stimulation [14]. Recently, Hausner et al. [15] showed a novel approach of accelerating regeneration after peripheral nerve injury, bridged with an autologous nerve graft. After dissecting and bridging the sciatic nerve of a Sprague-Dawley rat, extracorporeal shockwaves were applied at the site of injury. Six weeks after surgery, animals of the ESWT group exhibited a significantly
improved functional recovery relative to controls. On
the basis of this study, we investigated in vitro Schwann cell behavior after ESWT treatment with focus on their regenerative capacity.
Methods
Shockwave treatment of nerve tissue and Schwann
cell isolation
All animals were euthanized according to established protocols, which were approved by the City Government of Vienna, Austria, in accordance with the Austrian Law and Guide for the Care and Use of Laboratory Animals as defined by the National Institutes of Health. Animals and treatment/control groups were randomly chosen and analyzed without pre- or post-selection of the respective nerves or cultures. For ex vivo shockwave treatment an unfocused electro-hydraulic device was used (Dermagold 100, MTS Medical.The applicator was attached to a water bath as described in other studies [16–18], ensuring direct contact to the pre-warmed (37°C) water, allowing reproducible physical propagation and application of shockwaves in vitro. Sciatic nerves of adult male Sprague-Dawley rats were dissected, and each nerve was transferred into a 15-mL conical centrifuge tube (PAA Laboratories) containing phosphate buffered saline (PBS; PAA Laboratories) pre-chilled on ice. Nerves were kept on ice until further use but not longer than 1 h. For ESWT application, tubes were placed 5 cm in front of the applicator inside the water container. Subsequently, unfocused shockwaves were applied using the parameters chosen according to previous experiments [15] to maximize the effect of the ESWT treatment, while minimizing possible negative effects: 300 pulses at an energy level of 0.10 mJ/mm2 with a frequency of 3 Hz. The corresponding second nerve from the same animal served as control and was placed in a water bath (37°C) for the time of treatment to avoid the creation of artifacts due to different sample treatments. After ESWT treatment, Schwann cells were isolated from the treated and non-treated sciatic nerve tissues according to a method adapted from Kaewkhaw et al. [19]. Briefly, the epineurium was removed and nerves were weighed on a fine scale to assess nerve wet weight (Sartorius). Nerves were subsequently strained and minced. Nerve fragments were incubated with 0.05% collagenase (Sigma-Aldrich) for 1 h at 37°C and then filtered through a 40-μm cell strainer and centrifuged at 400g for 6 min. After washing the cell pellet with Dulbecco’s Modified Eagle Medium (DMEM; PAA Laboratories) containing 10% fetal calf serum (FCS; PAA Laboratories), the pellet was resuspended in DMEM-D-valine (PAA Laboratories), supplemented with 10% FCS, 2 mmol/L L-glutamine (PAA, Austria), 1% antibiotics (PAA Laboratories), N2 supplement (Invitrogen), 10 μg/mL bovine pituitary extract (Sigma-Aldrich), 5 μmol/L forskolin (Sigma-Aldrich). This medium is subsequently referred to as “Schwann cell medium.” Cell suspension was seeded on six-well plates (PAA Laboratories) coated with poly-L-lysin (Sigma-Aldrich) and laminin (Sigma-Aldrich).
Cell culture and experimental setup
Cells were subcultured for the first time after 19 days, to establish a proliferative phenotype and keep them in a proliferative state. Schwann cell medium was added on day 5 after isolation (1 mL) and was partially (50%) changed on days 9, 13 and 17. Subsequent splitting of cells was performed for 15 passages as follows: cells were detached with a cell scraper, centrifuged at 1200 rpm for 5 min and seeded at a density of 4 × 104 cells/cm2 on plates previously coated with poly-Llysin. Residual cells were used for flow cytometric analysis, 5-bromo-2-deoxyuridine uptake (BrdU) assay and protein isolation. Medium was partially (50%) changed every third day, and cells were split every sixth day.
Evaluation of cell yield
To evaluate cell yield, representative phase contrast pictures were taken from each culture using a Leica DMI6000B microscope (Leica), and cells were counted using a Bio-Rad TC20 automated cell counter (BioRad Laboratories). Non viable cells were identified and excluded by trypan blue staining. Cell count was normalized to 10-mg nerve wet weight assessed before isolation.
Flow cytometric analysis
Purity of the Schwann cell cultures was evaluated with flow cytometry for common Schwann cell markers: anti-S100b (rabbit polyclonal; Dako), anti-P75 NGFR (goat polyclonal; Santa Cruz Biotechnology) and antiP0 (rabbit polyclonal; Santa Cruz Biotechnology). Antibodies were labeled with allophycocyanin (Lynx Rapid Conjugation Kit, ABD Serotec). For analysis, cells were detached with a cell scraper and incubated with the antibodies (1:200) on ice and in the dark for 20 min. Cell pellets were washed twice and resuspended in 200 μL PBS. Flow cytometric analysis (10 000 events) was performed with a BD FACS Canto II (Becton Dickinson), and data were evaluated with Flowjo Version 8.8 (Tree Star).
Immunoblotting
Total protein of cells was extracted using Trizol (peqGold TriFast, Peqlab) according to manufacturer’s instructions. Briefly, proteins were precipitated from organic phase with ethanol and pelleted by centrifugation (12 000g, 10 min, 4°C). Protein pellet was washed three times with 0.3 mol/L guanidine hydrochloride (Sigma-Aldrich) in 95% ethanol and once with 100% ethanol (Merck), with each washing step followed by centrifugation (7500g, 5 min, 4°C).
Supernatants were discarded and dry protein pellets
solubilized in 1% SDS (Sigma-Aldrich) in analytical
grade water. Equal amounts of protein (up to 3 μg/lane; one
donor per gel: passage 2, passage 7, passage 15) were
separated on a 12% SDS-polyacrylamide gel and blotted onto a nitrocellulose membrane. Membranes were blocked with 5% skim milk inTris buffered saline containing 1% Triton-X100 (TBS-T; Sigma-Aldrich) for 120 min and incubated with primary antibodies S100b (Dako), c-Jun (Abcam), glial fibrillary acidic protein (GFAP; Bioss USA), P16INK4A (Abcam), α-tubulin (Calbiochem) diluted in 5% bovine serum
albumin (Sigma-Aldrich) in TBS-T at 4°C on a roll mixer for 12 h. Membranes were washed twice with TBS-T and incubated with the secondary antibody in 5% milk-TBS-T. Signals were detected using an Odyssey Fc infrared imaging system (LI-COR Biosciences). After membranes were incubated in 1 × NewBlot IR Stripping Buffer (LI-COR Biosciences) on a shaker at room temperature for 5 min and washed three times in PBS, membranes were reprobed
with total antibodies. Ratio of analyzed protein to housekeeping gene α-tubulin was densitometrically
analysed using Image Studio Version 5.0.21 (LICOR Biosciences).
Activation switch
In passages 4, 9 and 15, the activation status and the capacity to switch activation status (proliferating to promyelinating) were assessed. Cells were split (2 × 104 cells/cm2 , 24 h adherence time) in two groups: one was cultured in Schwann cell medium and the other in basic medium (DMEM-D-valine; PAA Laboratories), supplemented with 10% FCS, 2 mmol/L L-glutamine, 1% antibiotics) without supplements favoring the proliferating or the pro-myelinating status, respectively. Proliferation behaviour (BrdU enzymelinked immunosorbent assay) and marker expression (flow cytometry) were assessed 5 days after medium switch.
Adenosinetriphosphate release and lactate dehydrogenase release
The amount of adenosinetriphosphate (ATP) released into the supernatant from nerve tissue treated with ESWT was determined using the CellTiterGlo assay (Promega). Sciatic nerves were dissected and kept in PBS on ice until further treatment. After removing the epineurium and teasing of the nerve fibers with a mounted needle (15 times in the direction of the fiber), remaining nerve tissue was placed in 500 μL DMEM. Shockwave treatment was performed at 37°C and with following parameters: 300 pulses with 3 Hz and 0.03 mJ/mm2, 0.10 mJ/mm2 or 0.19 mJ/mm2. The control group was placed in a water bath (37°C). Nerve tissue was incubated for 5 min on ice and subsequently centrifuged at 1500 rpm for 5 min at 4°C. Supernatant was transferred to a micronic tube
(150 μL) for lactate dehydrogenase (LDH) measurement (Cobas C111; Roche Diagnostics) and a 96- well plate (triplicate, 100 μL) for ATP measurement. An equal amount of CellTiter-Glo reagent was added, the plate was horizontally shaken for 2 min, and after incubation for 10 min at room temperature, the resulting luminescence was measured. ATP standards were used for calibration of the measured luminescence. After sampling of the initial supernatant, fresh,
ice-chilled DMEM was added on nerve tissue and was
incubated on ice for another 30 min. ATP and LDH
concentration in supernatants was quantified as before.
Statistics
All data in this study are shown as mean ± SD and were tested for normal distribution. Depending on groups analyzed, statistical analysis was performed by using Student’s t-test or one-way analysis of variance followed by the Tukey range test for significant differences between the means. Significance was considered for P < 0.05. For statistical calculations, GraphPad Prism 5 for Mac OS X, Version 5.0b (GraphPad Software) was used.
Results
Increased cell yield by incorporation of ESWT in the
isolation process
To evaluate effects on the isolation efficacy, cells were counted after 19 days in culture, and cell number was normalized to 100 mg nerve wet weight. Sciatic nerve weights ranged between 66.2 and 88.5 mg, and there was no significant difference between the groups (Figure 1B). As shown in Figure 1A, cell yield after 19 days was significantly increased in the ESW treated group. Although initial cell count revealed 1.62 × 106 ± 9.3% cells per 100 mg nerve wet weight in the control group, cell yield in the ESW-treated group was, on average, 52.3% higher (3.10 × 106 ± 8.2% cells per 100 mg nerve wet weight). Furthermore, Figure 2A illustrates a consistent improvement of the cell yield for every culture assessed.
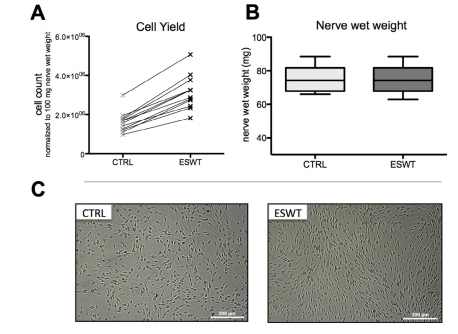
normalized on 100 mg nerve wet weight, n = 12. (B) nerve wet weight of the respective nerves, assessed with a fine scale before
isolation; n = 12. (C) Phase contrast micrographs of SCs in passage 0, untreated control (CTRL) and treated with extracorporeal shockwaves
(ESWT).
Proliferation assay
Proliferation assay using a BrdU (Cell Proliferation ELISA Assay Kit; Roche Diagnostics) for quantitative evaluation of Schwann cell proliferation was performed according to manufacturer’s instructions. Briefly, poly-L-lysine coated 96-well plates were seeded with cells at a density of 2.5 × 104 cells/cm2. After 48 h, medium was changed to Schwann cell medium containing 100 μmol/L BrdU, and cells were incubated for 24 h at standard cell culture conditions (37°C and 5% CO2). The culture plates were fixated with FixDenat solution and subsequently incubated with anti-BrdU POD antibody solution for 60 min at room temperature. After washing the plate with PBS, tetramethyl benzidine was added for 30 min as a substrate.The reaction was stopped with 1 mol/L H2SO4 and absorption was measured at 450 nm with 690 nm as reference wavelength on an automatic microplate reader (Tecan Sunrise).
BrdU assay and population doublings per passage reveal a significantly higher proliferation after ESWT for 15 passages
The cell proliferation was quantified using a BrdU assay and assessment of population doublings per passage. In all passages examined (passage 1, 4, 7, 10, 13 and 15), Schwann cells treated with ESWT showed a higher proliferative behavior than the Schwann cells in the control group, respectively (Figure 2). Furthermore proliferation decreased steadily in the control group starting in passage 4, whereas proliferation in the ESWT group further increased until passage 7 and remained at a similar level until passage 15. Western blots in passage 2, 7 and 15 also exhibited a steady increase in the cell-cycle-arrest/senescence marker P16INK4a in the control group, while ESWT group remained at the same low level for the tested passages (Figure 4).
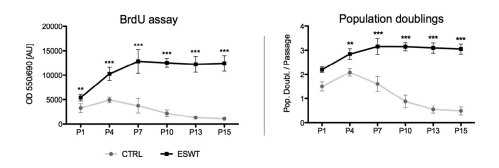
Sustained purity and P75 expression after ESWT for
extended culture period
To determine purity and phenotype of these cultivated cells, expression of Schwann cell specific marker S100b, as well as the markers P75 (proliferative/regenerative phenotype) and P0 (myelinating phenotype), were assessed in vitro over 15 passages with flow cytometry (Figure 3). In passage 1, 24.2% more cells expressed S100b in the ESW-treated group compared with the control group (62.6% compared with
38.4%). In both groups, Schwann cell purity increased in passage 2 to 70.0% (ESWT) and 57.3% (CTRL). However, starting in passage 3, S100b expression in the control group decreased steadily over the following passages. In contrast, in the ESW treated group expression increased until passage 5 (85.3%) and remained at this level until passage 15 (Figure 3,
S100b). A similar temporal pattern was applied to the expression of the proliferation-associated marker P75: while control group reached a peak expression of 36.7% in passage 3, steadily decreasing over time, ESW-treated cells increased from 57.4% P75 expression to 83.1% positive cells and remained at this level until passage 15 (Figure 3, P75). Myelin marker P0 was down-regulated in both groups; however, it was stronger in the ESWT treated group, exhibiting a significant difference in passage 3 where control group showed an increase of 9.0% compared to passage 2 (Figure 3, P0). Protein expression levels usingWestern blot densitometric analysis revealed a similar difference between the groups in the expression of S100b as found with flow cytometry in the respective passages passage 2, 7 and 15 (Figure 4, S100). Further analysis of the markers c-Jun and GFAP, both associated with the proliferative Schwann cell phenotype, exhibited an increase of both markers in the ESWT group and a decrease in the control group (Figure 4).
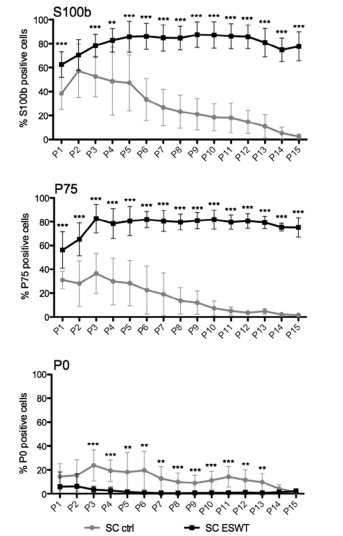
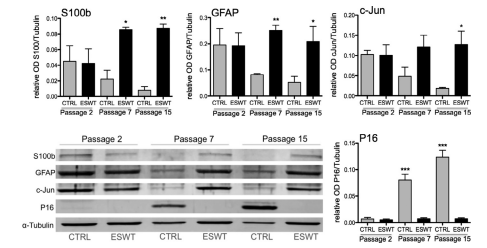
Schwann cells treated with ESWT display a consistent morphology
Schwann cell morphology was assessed in passage 0, 5, 9 and 15 using phase contrast microscopy (Figure 5). Schwann cells of both groups showed a spindle or tripolar shape in passage 0. Starting in passage 5, a second cell morphology was found in the control group, similar to the classic fibroblastic phenotype. Cells exhibiting this morphology increased in number from passage 5 to passage 15 in the control group (Figure 5, indicated with arrows), whereas the ESWT-treated group revealed a homogenous Schwann cell morphology over 15 passages.
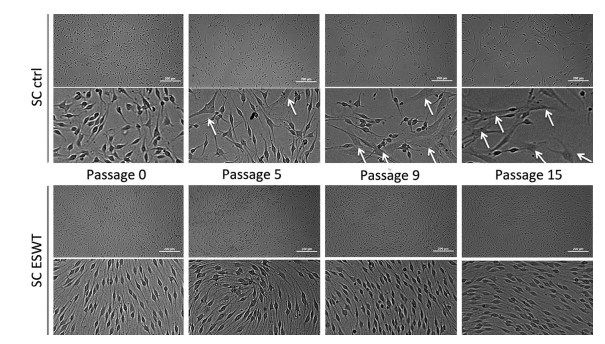
extracorporeal shockwaves (ESWT). Phase contrast micrographs depicting Schwann cells in passage 0, 5, 9 and 15 of control group (CTRL,
upper bar) and ESW-treated group (lower bar); arrows mark cells displaying non-typical morphology (tripolar, fibroblast-like); size bar
indicates 200 μm.
Schwann cells revert faster to myelinating phenotype after ESWT To demonstrate that the proliferative activation of SCs is not permanent and reversible the Schwann cell ability to switch phenotype from proliferating to promyelinating was tested.Therefore, in passages 4, 9 and 15, the medium was changed to one lacking proproliferative growth factors, giving the cells a minimal stimulus to change their phenotype. Flow cytometry revealed no change in expression of S100b between
the cells cultured in the different mediums in the respective passages (Figure 6). In passage 4, cells of the control group and the ESWT group were able to perform the switch, as can be seen in Figure 6: P75 was significantly down-regulated, whereas P0 was strongly up-regulated. Expression of P0, however, was significantly higher in the ESW-treated group. In passages 9 and 15 only, cells in the ESWT group reacted to the stimulus by significant down-regulation of P75 together with up-regulating P0. BrdU assay confirmed a significantly decreased proliferation in the ESW-treated group cultured in pro-myelination medium compared with proliferation medium (Figure 6, lower panel).
ESWT induces immediate and sustained ATP release
Purinergic signaling is capable of inducing enhanced proliferation after ESWT treatment, associated with ATP release [18].Therefore, we assessed ATP release of the ex vivo–treated sciatic nerves. Extracellular concentration of ATP was enhanced depending on the applied energy flux densities. The “minimal” setting with the lowest energy level (0.03 mJ/mm2) did not result in any initial increase, whereas application of ESW with treatment (0.1 mJ/mm2 ) as well as the “maximal” setting (0.19 mJ/mm2) had a significant increase in initial extracellular ATP as a consequence (Figure 7, ATP 5 min). Nerve tissue was incubated in freshly added DMEM for 30 min on ice to assess sustained ATP release. These supernatants showed a generally lower concentration of ATP in all groups; however, treatment (0.1 mJ/mm2) and maximal setting (0.19 mJ/mm2) again showed higher levels than control and minimal setting (0.03 mJ/mm2; Figure 7, ATP 30 min). Among other triggers, ATP can be released into the extracellular space due to cell membrane damage.To exclude membrane damage as the reason for increased extracellular ATP after ESW treatment we assessed extracellular LDH concentration as an indicator for cell damage. As seen in Figure 7 (LDH), supernatants of nerve tissue that received the highest energy (0.19 mJ/mm2) were the only ones that showed
significantly enhanced LDH concentrations. At both time points, our selected treatment setting (0.1 mJ/mm2) did not increase levels of extracellular LDH when compared with the control group.
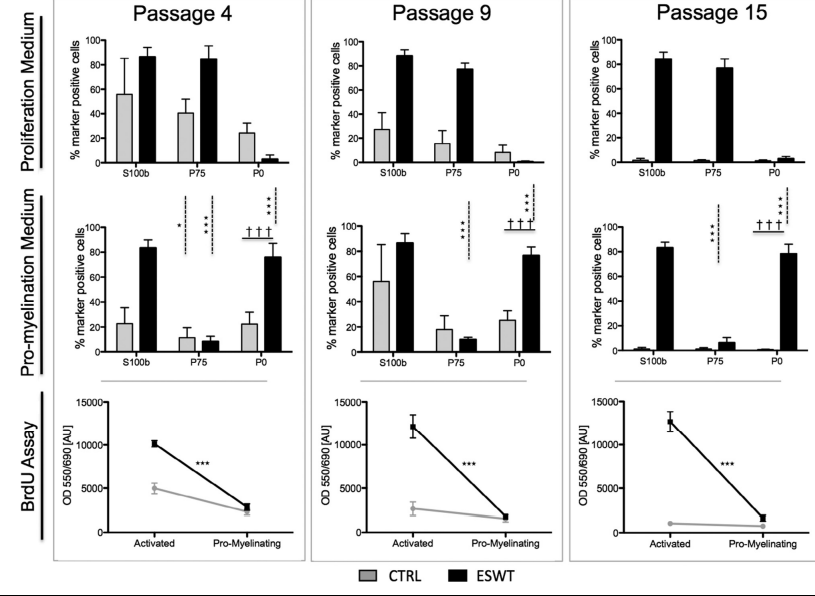
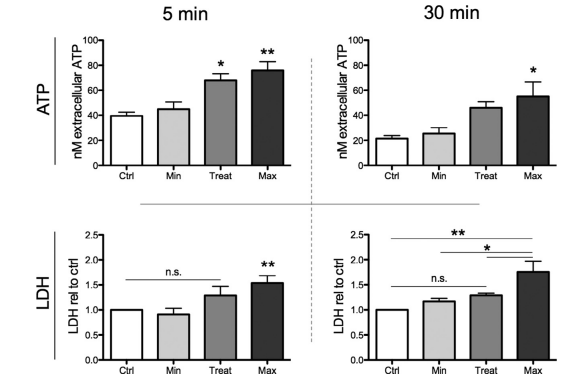
Discussion
After peripheral nerve injury, Schwann cells are triggered to change their phenotype from myelinating to activated and proliferating and building bands of Büngner, the substrate for outgrowing axons. There are myriad studies describing the importance of Schwann cells during peripheral nerve regeneration [20–22], but the difficulties and issues associated are also well known [23–25]. Particularly in long-distance injuries, the demand for supportive Schwann cells expanded in vitro (e.g., seeded on a tubular graft) would be high because autologous Schwann cells lack the proliferative capacity to provide bands of Büngner throughout a tube longer than 40 mm [5].This limited proliferative capacity is also reflected in vitro, displaying together with insufficient purity the main issue of Schwann cell cultures. To our knowledge, we are the
first to demonstrate an increase in proliferation, proliferative capacity and purity in in vitro Schwann cell cultures with ESWT. The basis for our study was found in two preceding shockwave studies. Hausner et al. [15] showed that shockwave treatment after nerve dissection leads to significantly accelerated regeneration in a rat model. It was hypothesized that this effect may result from improved macrophage infiltration and an earlier onset of regeneration. However,Weihs et al. [18] elucidate in their study how ESWT stimulates cell proliferation in several cell types (e.g., mesenchymal cells) by ATP release-coupled extracellular signal-regulated kinase (ERK) activation. Applying ESWT on the whole nerve before isolation of Schwann cells, our results
demonstrate that ESWT is capable of enhancing the extracellular levels of ATP without causing any cell
membrane damage. Furthermore, the sustained release
over 30 min suggests an active mechanism of ATP release. Analyzing the cultures in passage 0, we assessed a significantly higher cell yield in all cultures treated with ESWT (Figure 1), alongside a significantly increased proliferation rate in passage 1 (Figure 2). In the shockwave-treated group, quality of culture, reflected in purity and proliferation rate, improved over the first passages: S100b, a marker indicating purity of Schwann cells, increased over the first five passages and remained at the level for the period of analysis; this purity was also observed in morphology of Schwann cells (Figure 4); moreover, P75 increased over time, whereas P0 in the same manner decreased until passage 4 and subsequently was not expressed (Figure 3); P0 and P75 are known to counteract each other [26]: P0 is a myelin component and therefore solely expressed in myelinating Schwann cells [27], whereas P75 is a marker associated with the regenerative, proliferating phenotype of Schwann cells
[28,29]. Western blot analysis at three time points throughout the culture period revealed a similar expression pattern for S100b, acting as a control marker between the methods (Figure 4, compared with Figure 5 S100b). GFAP and c-Jun were also up-regulated in passage 7 (compared with passage 2) and showed a comparable expression in passage 15. Both markers are associated with the regenerative phenotype. GFAP
is suppressed in myelinated axons and becomes upregulated after injury, initiating proliferation by binding of integrin αvβ8 [30]. The transcription factor c-Jun however is an antagonist to Krox20, a protein controlling the myelination in the peripheral nervous system, and displays one of the key regulators to initiate and maintain the regenerative phenotype [31,32].
Together with the strongly increased proliferation (assessed with BrdU assay and population doubling per passage), the Schwann cells treated with ESWT represent a highly regenerative phenotype for an extended culture period of 15 passages (103 days). As the sum of these observations leads inevitably to the question whether hyper-proliferating Schwann cells may lead to adverse effects such as post-stimulus proliferation and subsequently Schwannoma formation, we conducted a functionality experiment. Schwann cells were cultured for 5 days in basic medium lacking any kind of proliferation stimulating growth factors (forskolin, pituitary extract). Schwann cells treated with ESWT reacted even more to the change of stimulus, independent of the passage: they not only stopped proliferating (Figure 6, BrdU)
but also significantly down-regulated the proliferationassociated marker P75 and up-regulated the myelin component P0. This prompt and consistent reaction
to the absence of mitogenic growth factors demonstrates their capacity to switch to the myelinating phenotype. However, the functionality of the SCs in an in vivo defect model has to be shown. In contrast to the ESWT group, the untreated
Schwann cells did not display a consistent Schwann cell phenotype over the culture period of 15 passages. Starting with passage 5 to 7, purity and
proliferation significantly decreased. Abated purity was
observed in lower expression of S100b (Figures 3 and
6), as well as in increased appearance of cells displaying an atypical morphology (Figure 5). Reduced
proliferation was primarily assessed in a decline of population doublings and additionally by reduced BrdU
OD values.The entire marker expression levels associated with the regenerative phenotype (P75, GFAP,
c-Jun) diminished over time, whereas the senescence marker P16 significantly increased. Therefore,
our in vitro study reflects the before mentioned in vivo
problem and represents a possible solution: the limited
proliferative capacity of Schwann cells can be improved with ESWT. With treated Schwann cells
building a growth substrate faster and for a longer
period of time, the in vivo effect can be twofold: ESWT
not only results in an acceleration of regeneration
by activating autologous Schwann cells, as has been
shown by Hausner et al. [15], but would also allow
reimplantation of a high number of autologous
Schwann cells expanded ex vivo in a decidedly regenerative state. A partial explanation for the underlying mechanisms of the observed effects could be the sustained
ATP release. A wide range of mechanisms from vesicular release over connexins/pannexins to ABC
transporters are thought to conduct an active release
of ATP [33–35]. Subsequent purinergic signaling
plays a crucial role not only as a danger-associated
molecular pattern but also in a variety of cellular functions such as proliferation, chemotaxis, differentiation
and amplification of other signals [18,36]. This includes Schwann cell–axon interactions. Immature/
unmyelinating Schwann cells in particular signal via
extracellular ATP in a paracrine manner with axons
[37,38]. It was proposed that ATP and glutamate are
building a positive feedback loop enhancing their activities [39]. The fate of Schwann cells is influenced
by neuronal activity, by the activation of purinergic
metabotropic P2Y receptors and direct actions of ATP
and its metabolite adenosine [40–42], as well as by
the activation of metabotropic glutamate receptors
[43]. Furthermore, purinergic signaling is thought to
be both an autocrine and a paracrine amplifier for
other signaling inputs. This is a further explanation
for the enhanced proliferative activity of ESWTtreated Schwann cells in medium-containing
proliferation-inducing factors such as forskolin and
pituitary extract.
Adenosine, a metabolic product of
ATP hydrolization, is hypothesized to play a role in
learning by affecting histone-modifying proteins resulting in epigenetic changes [44]. Therefore, the
prolonged phenotypic stability and increased susceptibility to external stimuli as observed in the phenotype switch experiments in this study may be explained
by epigenetic changes.
In conclusion, we observed a higher proliferative
activity without phenotype commitment, increased
purity of culture and reduced expression of senescenceassociated markers even after long cultivation periods. These positive effects of ESWT on Schwann cell isolation and cultivation may partly be explained by the
actions of extracellular ATP. However, to gain a deeper
understanding of the effects of ESWT on Schwann
cells and their natural habitat, the nerve, additional
in vitro and in vivo studies focusing on purinergic signaling, mechanotransduction and epigenetic processes
have to be performed.
Acknowledgments
The financial support by the City of Vienna Project
(MA23#14-06) is gratefully acknowledged.We thank
Anna Khadem for assistance with the LDH measurement and Susanne Wolbank for proofreading the
manuscript. Disclosure of interest: The authors have no commercial, proprietary, or financial interest in the products
or companies described in this article.
References
[1] Mukhatyar V, Karumbaiah L, Yeh J, Bellamkonda R. Tissue
engineering strategies designed to realize the endogenous
regenerative potential of peripheral nerves. Adv Mater
2009;21:4670–9.
[2] Siemionow M, Brzezicki G. Chapter 8: current techniques and
concepts in peripheral nerve repair. Int Rev Neurobiol
2009;87:141–72.
[3] Arino H, Brandt J, Dahlin LB. Implantation of Schwann cells
in rat tendon autografts as a model for peripheral nerve repair:
long term effects on functional recovery. Scand J Plast
Reconstr Surg Hand Surg 2008;42:281–5.
[4] Johnson EO, Soucacos PN. Nerve repair: experimental and
clinical evaluation of biodegradable artificial nerve guides.
Injury 2008;39:29–33.
[5] Saheb-Al-Zamani M, Yan Y, Farber SJ, Hunter DA, Newton
P, Wood MD, et al. Limited regeneration in long acellular
nerve allografts is associated with increased Schwann cell
senescence. Exp Neurol 2013;247:165–77.
[6] Chen Z-L, Yu W-M, Strickland S. Peripheral regeneration.
Annu Rev Neurosci 2007;30:209–33.
[7] Kaude JV, Williams CM, Millner MR, Scott KN, Finlayson
B. Renal morphology and function immediately after
extracorporeal shock-wave lithotripsy. AJR Am J Roentgenol
1985;145:305–13.
[8] Schaden W, Fischer A, Sailler A. Extracorporeal shock wave
therapy of nonunion or delayed osseous union. Clin Orthop
Relat Res 2001;90–4.
[9] Furia JP, Juliano PJ, Wade AM, Schaden W, Mittermayr R.
Shock wave therapy compared with intramedullary
screw fixation for nonunion of proximal fifth metatarsal
metaphyseal-diaphyseal fractures. J Bone Jt Surg Am
2010;92:846–54.
[10] Elster EA, Stojadinovic A, Forsberg J, Shawen S, Andersen
RC, Schaden W. Extracorporeal shock wave therapy for
nonunion of the tibia. J Orthop Trauma 2010;24:133–41.
[11] Mittermayr R, Hartinger J, Antonic V, Meinl A, Pfeifer S,
Stojadinovic A, et al. Extracorporeal shock wave therapy
(ESWT) minimizes ischemic tissue necrosis irrespective of
application time and promotes tissue revascularization by
stimulating angiogenesis. Ann Surg 2011;253:1024–32.
[12] Schaden W, Thiele R, Kölpl C, Pusch M, Nissan A, Attinger
CE, et al. Shock wave therapy for acute and chronic soft tissue
wounds: a feasibility study. J Surg Res 2007;143:1–12.
[13] Saggini R, Figus A, Troccola A, Cocco V, Saggini A, Scuderi
N. Extracorporeal shock wave therapy for management of
chronic ulcers in the lower extremities. Ultrasound Med Biol
2008;34:1261–71.
[14] Ogden JA, Tóth-Kischkat A, Schultheiss R. Principles of shock
wave therapy. Clin Orthop Relat Res 2001;8–17.
[15] Hausner T, Pajer K, Halat G, Hopf R, Schmidhammer R,
Redl H, et al. Improved rate of peripheral nerve regeneration
induced by extracorporeal shock wave treatment in the rat.
Exp Neurol 2012;236:363–70.
[16] Holfeld J, Tepeköylü C, Kozaryn R, Urbschat A, Zacharowski
K, Grimm M, et al. Shockwave therapy differentially stimulates
endothelial cells: implications on the control of inflammation
via toll-like receptor 3. Inflammation 2014;37:65–70.
[17] Schuh CMAP, Heher P, Weihs AM, Banerjee A, Fuchs C,
Gabriel C, et al. In vitro extracorporeal shock wave treatment
enhances stemness and preserves multipotency of rat and
human adipose-derived cells. Cytotherapy 2014;16:
1666–78.
[18] Weihs A, Fuchs C, Teuschl A, Hartinger J, Slezak P,
Mittermayr R, et al. Shock wave treatment enhances cell
proliferation and improves wound healing by ATP releasecoupled extracellular signal-regulated kinase (ERK) activation.
J Biol Chem 2014;27090–104.
[19] Kaewkhaw R, Scutt AM, Haycock JW. Integrated culture and
purification of rat Schwann cells from freshly isolated adult
tissue. Nat Protoc 2012;7:1996–2004.
[20] Frostick SP, Yin Q, Kemp GJ. Schwann cells, neurotrophic
factors, and peripheral nerve regeneration. Microsurgery
1998;18:397–405.
[21] Toy D, Namgung U. Role of glial cells in axonal regeneration.
Exp Neurobiol 2013;22:68–76.
[22] Bhatheja K, Field J. Schwann cells: origins and role in axonal
maintenance and regeneration. Int J Biochem Cell Biol
2006;38:1995–9.
[23] Casella GTB, Bunge RP, Wood PM. Improved method for
harvesting human Schwann cells from mature peripheral nerve
and expansion in vitro. Glia 1996;17:327–38.
[24] Thi AD, Evrard C, Rouget P. Proliferation and differentiation
properties of permanent Schwann cell lines immortalized with
a temperature-sensitive oncogene. J Exp Biol 1998;201:851–
60.
[25] Lehmann HC, Chen W, Mi R, Wang S, Liu Y, Rao M, et al.
Human schwann cells retain essential phenotype characteristics
after immortalization. cells Dev 2012;21:423–31.
[26] Lemke G, Chao M. Axons regulate Schwann cell expression
of the major myelin and NGF receptor genes. Development
1988;102:499–504.
[27] Lemke G. Molecular biology of the major myelin genes.
Trends Neurosci 1986;9:266–70.
[28] Jessen KR, Mirsky R. The origin and development of
glial cells in peripheral nerves. Nat Rev Neurosci 2005;6:671–
82.
[29] Provenzano MJ, Minner SA, Zander K, Clark JJ, Kane CJ, Green SH, et al. p75(NTR) expression and nuclear localization of p75(NTR) intracellular domain in spiral ganglion Schwann cells following deafness correlate with cell proliferation. Mol
Cell Neurosci 2011;47:306–15.
[30] Triolo D, Dina G, Lorenzetti I, Malaguti M, Morana P,
Del Carro U, et al. Loss of glial fibrillary acidic protein
(GFAP) impairs Schwann cell proliferation and delays nerve
regeneration after damage. J Cell Sci 2006;119:3981–93.
[31] Arthur-Farraj PJ, Latouche M, Wilton DK, Quintes S, Chabrol
E, Banerjee A, et al. c-Jun reprograms schwann cells of injured
nerves to generate a repair cell essential for regeneration.
Neuron 2012;75:633–47.
[32] Parkinson DB, Bhaskaran A, Arthur-Farraj P, Noon LA,
Woodhoo A, Lloyd AC, et al. c-Jun is a negative regulator of
myelination. J Cell Biol 2008;181:625–37.
[33] Schwiebert EM, Zsembery A. Extracellular ATP as a signaling
molecule for epithelial cells. Biochim Biophys Acta 2003;
1615:7–32.
[34] Abbracchio MP, Burnstock G, Boeynaems J-M, Barnard EA,
Boyer JL, Kennedy C, et al. International Union of
Pharmacology LVIII: update on the P2Y G protein-coupled
nucleotide receptors: from molecular mechanisms and
pathophysiology to therapy. Pharmacol Rev 2006;58:281–341.
[35] Lazarowski ER. Vesicular and conductive mechanisms of
nucleotide release. Purinergic Signal 2012;8:359–73.
[36] Junger WG. Immune cell regulation by autocrine purinergic
signalling. Nat Rev Immunol 2011;11:201–12
[37] Liu GJ, Bennett MR. ATP secretion from nerve trunks
and Schwann cells mediated by glutamate. Neuroreport
2003;14:2079–83.
[38] Samara C, Poirot O, Domènech-Estévez E, Chrast R.
Neuronal activity in the hub of extrasynaptic Schwann
cell-axon interactions. Front Cell Neurosci 2013;7:
228.
[39] Jeftinija SD, Jeftinija KV. ATP stimulates release of excitatory
amino acids from cultured Schwann cells. Neuroscience
1997;82:927–34.
[40] Stevens B, Fields RD. Response of Schwann cells to action
potentials in development. Science 2000;287:2267–
71.
[41] Fields RD, Burnstock G. Purinergic signalling in neuron-glia
interactions. Nat Rev Neurosci 2006;7:423–36.
[42] Stevens B, Ishibashi T, Chen J-F, Fields RD. Adenosine: an
activity-dependent axonal signal regulating MAP kinase and
proliferation in developing Schwann cells. Neuron Glia Biol
2004;1.
[43] Saitoh F, Araki T. Proteasomal degradation of glutamine
synthetase regulates schwann cell differentiation. J Neurosci
2010;30:1204–12.
[44] Boison D, Singer P, Shen H-Y, Feldon J, Yee BK. Adenosine
hypothesis of schizophrenia—opportunities for pharmacotherapy. Neuropharmacology 2012;62:1527–43.